High-temperature superconductors are layered materials with complex chemical structures involving transition metals like copper or iron, oxygen, and specific dopants that enable zero electrical resistance at relatively high temperatures. By carefully adjusting their composition and doping levels, you can enhance their superconducting properties. Their layered crystal structures create anisotropic electrical pathways, while electron pairing mechanisms involving spin fluctuations or charge transfer explain how resistance drops. To discover more about these fascinating materials, explore how their chemistry unlocks zero resistance.
Key Takeaways
- High-temperature superconductivity arises from layered cuprate and iron-based materials with specific chemical compositions and oxygen content.
- Doping alters electronic properties, promoting electron pairing and increasing critical temperatures in these complex compounds.
- Crystal structures feature CuO₂ planes or iron layers that facilitate anisotropic conductivity and superconducting behavior.
- Magnetic interactions, such as spin fluctuations, play a crucial role in electron pairing mechanisms leading to zero resistance.
- Achieving high Tc involves precise chemical control, including element substitution and oxygen doping, to optimize electronic and lattice interactions.
The Composition of High-Temperature Superconducting Materials
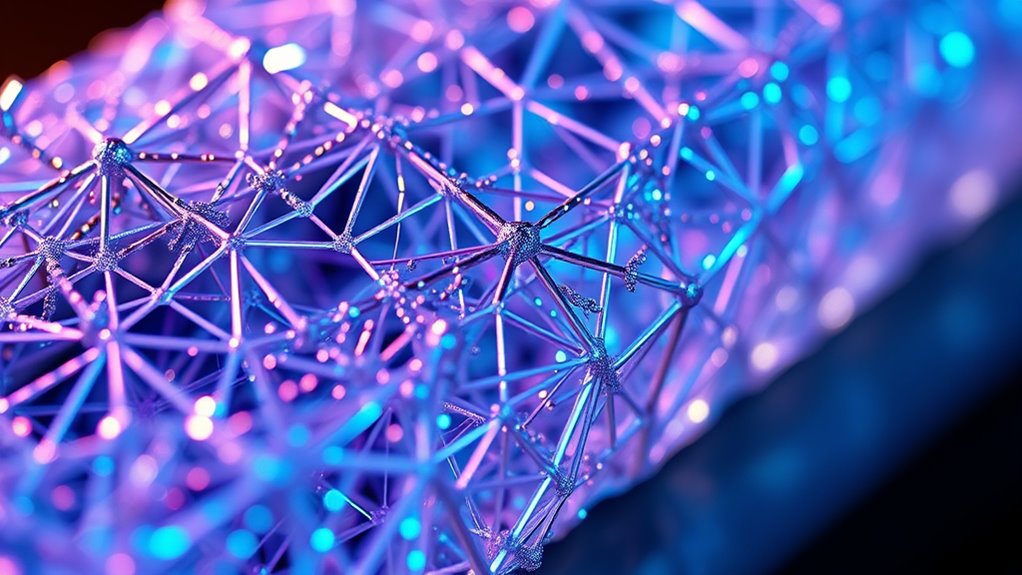
High-temperature superconductors are primarily composed of layered materials containing transition metals and oxygen, where specific elemental constituents determine their superconducting properties. You’ll find copper oxides, or cuprates, as the main component, especially in well-studied high-T_c materials like YBCO, which includes yttrium, barium, copper, and oxygen. These compounds’ properties depend heavily on their oxygen content and doping levels, which you can tweak to optimize superconductivity. Doping levels are often adjusted through chemical substitution or oxygenation processes. Iron-based superconductors, featuring layers of iron arsenide or selenide, offer another class with distinct electronic traits. Nickel compounds are also explored for their similarities to copper oxides, providing insights into high-T_c phenomena. Oxygen’s role is vital across all these materials, affecting their crystal chemistry and the critical temperature at which they become superconducting.
Crystal Structures and Layered Motifs Enabling Superconductivity
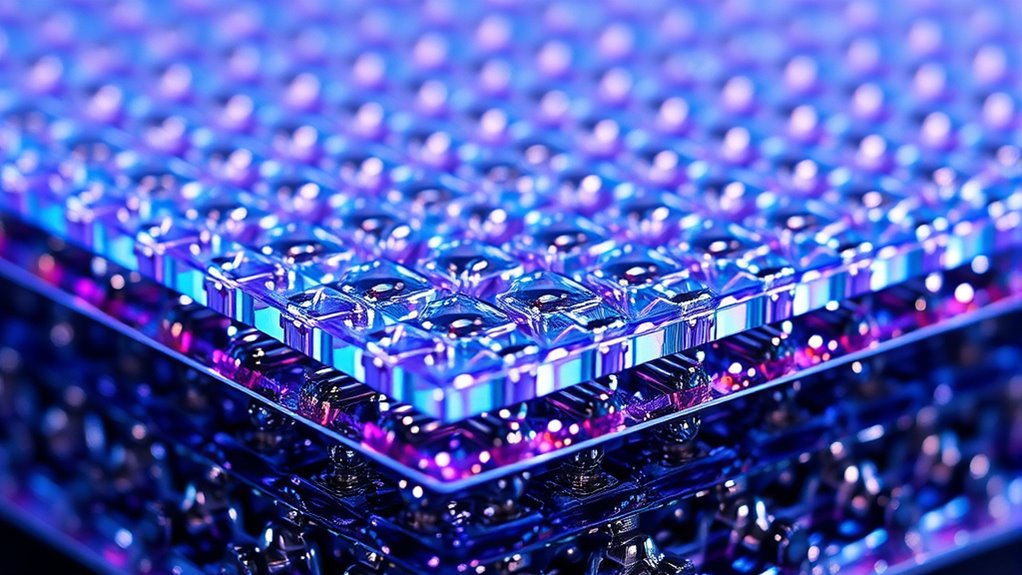
The unique crystal structures of high-temperature superconductors underpin their ability to conduct electricity without resistance at elevated temperatures. These structures often resemble distorted, oxygen-deficient multilayered perovskite frameworks, with alternating CuO2 planes forming the core motif for superconductivity. Indexing blockchain data facilitates understanding of the complex structural relationships within these materials. The number of CuO2 layers, denoted by n, influences the critical temperature, with higher n generally leading to increased Tc. Spacer layers, like yttrium planes in YBCO, affect layer coupling and electronic properties, while Cu–O chains enhance superconductivity. The crystal systems can be orthorhombic or tetragonal, with oxygen content dictating phase stability and Tc. The layered architecture causes anisotropic electrical conductivity, with high conduction within CuO2 planes and limited flow perpendicular to them, mediated by Josephson coupling across layers.
Achieving and Enhancing Superconductivity Through Chemical Doping
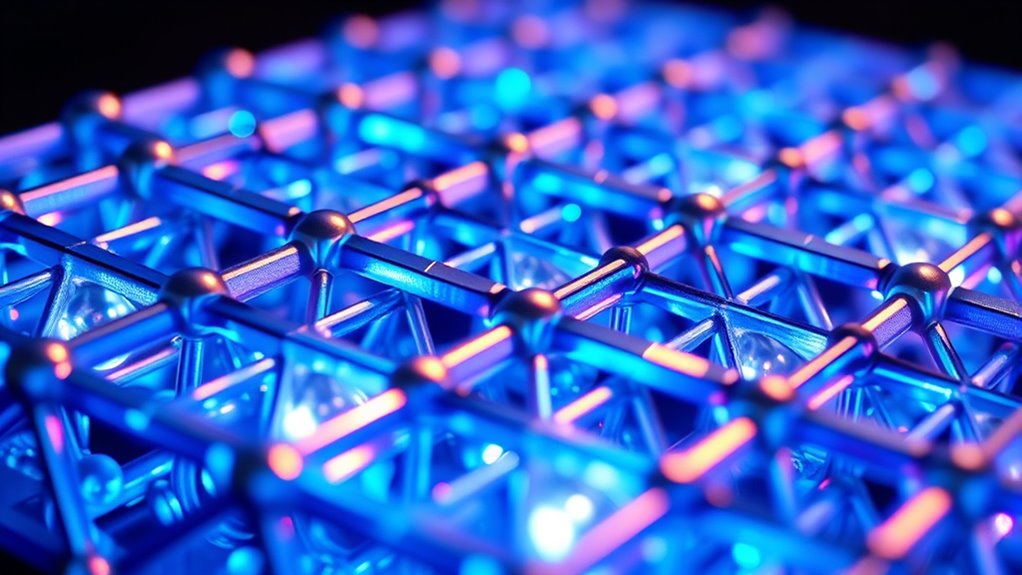
Chemical doping plays a crucial role in achieving and enhancing superconductivity in layered materials by modifying their electronic and lattice properties. By substituting elements like rare-earth or transition metals at specific lattice sites, you can influence electron density and electron-phonon interactions, which directly impact the superconducting transition temperature (Tc).
For example, Re doping in Mo5Si3 increases Tc up to 7.7 K, while site-selective arsenic doping achieves similar improvements. Doping typically causes phonon mode softening, boosting electron-phonon coupling essential for superconductivity.
However, magnetic impurities like Fe can rapidly suppress Tc through magnetic scattering, aligning with the Abrikosov-Gorkov theory. Overall, strategic doping can either enhance or suppress superconductivity, depending on the dopant type, concentration, and its effect on lattice dynamics.
Transition Temperatures and Notable Material Examples
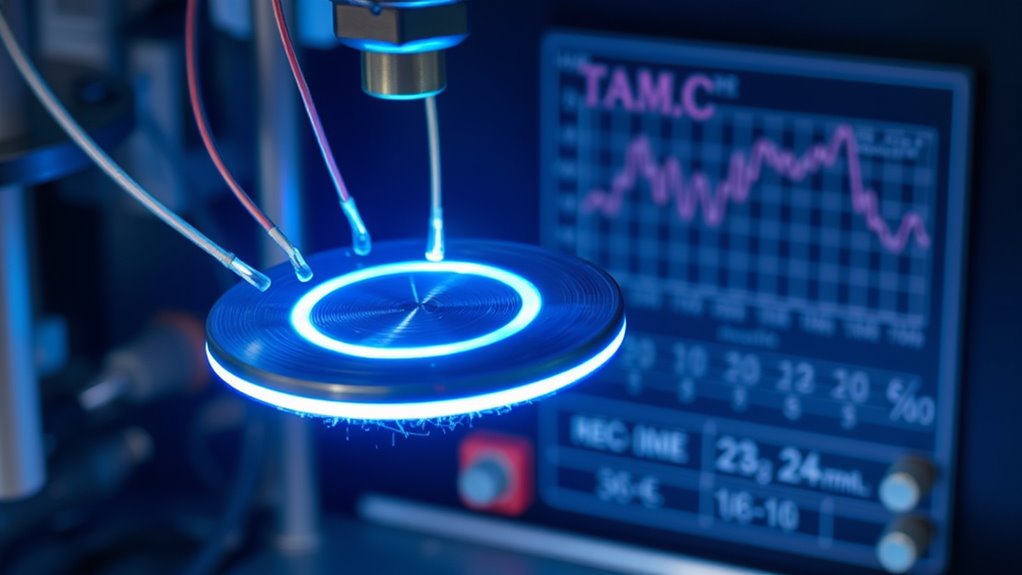
Understanding how different materials behave as they approach their superconducting state helps you appreciate the diverse range of transition temperatures observed across compounds.
Conventional superconductors usually have Tc between 1 K and 20 K, with tungsten at 0.015 K and niobium reaching 9.2 K.
Cuprates show much higher Tc values, often above 90 K, and some mercury-based cuprates hit around 133 K at ambient pressure.
Hydrogen sulfide under high pressure achieves about 203 K, though this isn’t at normal conditions.
Recent discoveries suggest possible superconductivity near 250 K under extreme pressures.
Notable materials include YBCO with Tc above 90 K, mercury cuprates at 133 K, and iron-based superconductors around 30–55 K.
These variations highlight how chemical composition, pressure, and structure influence superconducting transition temperatures.
Underlying Mechanisms and Theoretical Insights Into Electron Pairing
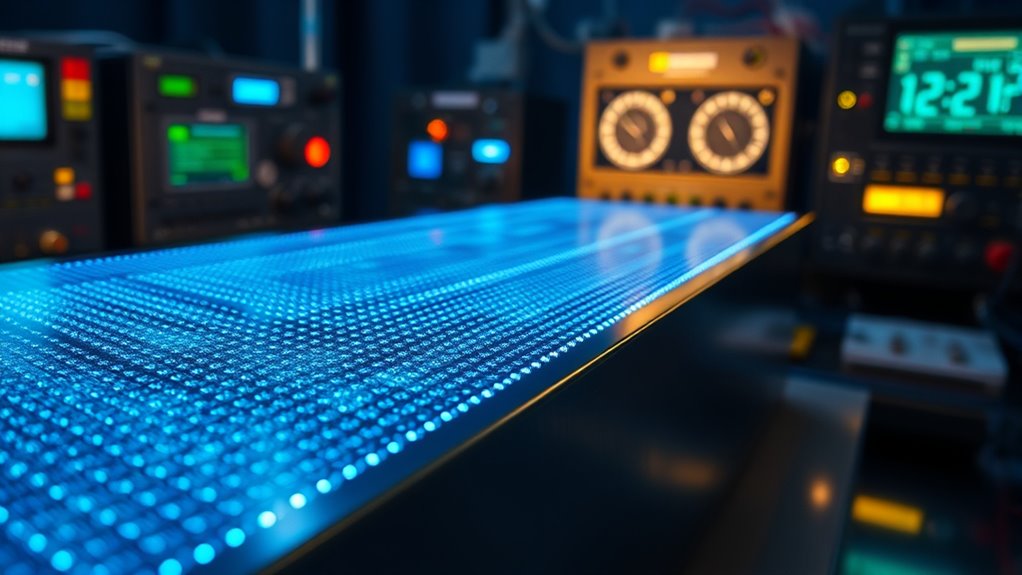
Exploring the mechanisms behind electron pairing in high-temperature superconductors reveals a complex interplay of magnetic and electronic interactions. You’ll find theories like spin fluctuation, where short-range spin waves (paramagnons) mediate pairing, and charge-transfer superexchange, which involves electron interactions across energy gaps. Electron-electron interactions, influenced by strong correlations, also play a role. High-temperature superconductivity often emerges from an antiferromagnetic state, disrupted by hole doping but maintaining pair-forming interactions. Quantum rules allow Cooper pairs to overlap, creating zero resistance. Below is a table summarizing key mechanisms:
Mechanism | Key Feature |
---|---|
Spin Fluctuation | Mediated by paramagnons, short-range spin waves |
Charge-Transfer Superexchange | Electron interactions across charge-transfer gaps |
Electron-Electron Interaction | Driven by strong correlations, electron repulsion |
Antiferromagnetic State | Disrupted by doping, preserves pairing interactions |
Quantum Mechanical Rules | Cooper pairs act as overlapping quantum particles |
Additionally, understanding magnetic interactions is essential to fully grasp the pairing mechanisms in these materials.
Practical Applications and Future Directions in Superconducting Technology
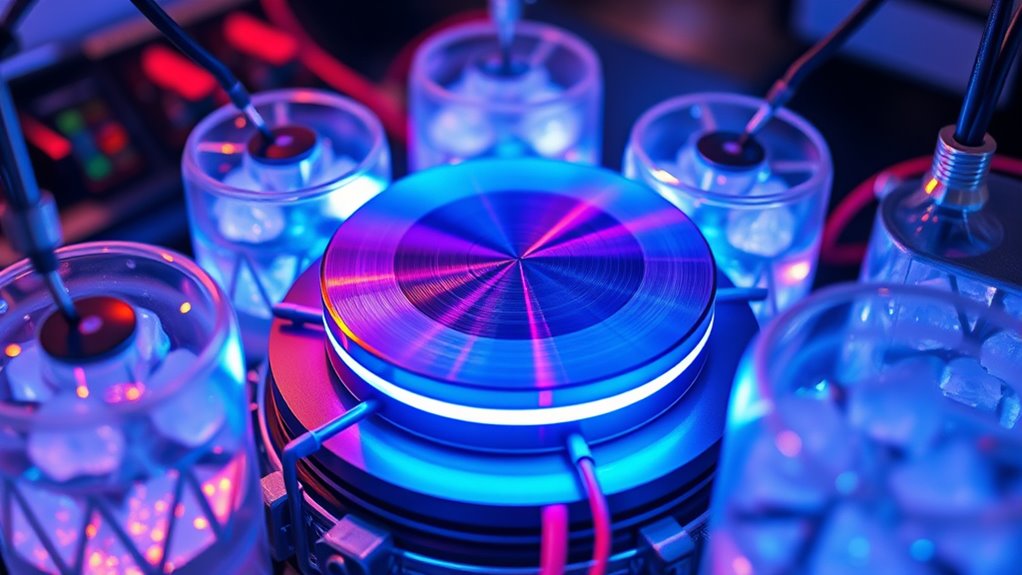
High-temperature superconductors have moved beyond their theoretical foundations to become key components in modern technology. You benefit from their use in power systems, where HTS cables reduce energy loss and fault current limiters enhance grid stability. Superconducting magnetic energy storage (SMES) provides efficient energy backup, while HTS-powered motors and generators improve efficiency and cut weight.
In medical imaging, MRI machines rely on superconductors for high-quality imaging, and particle accelerators use HTS magnets to achieve higher magnetic fields at lower costs. Future development focuses on improving durability, critical current densities, and higher critical temperatures, which could reduce cooling needs. Ongoing research continues to improve the material properties and manufacturing techniques of HTS, leading to broader adoption and more innovative applications. Advances in fabrication and integration will expand applications, making superconducting tech more practical, cost-effective, and essential across industries.
Frequently Asked Questions
What Are the Main Challenges in Synthesizing High-Temperature Superconductors?
You face numerous challenges when synthesizing high-temperature superconductors. Controlling complex, multicomponent crystal structures requires precise conditions, and maintaining phase purity is tough.
High pressures and extreme environments are often necessary, making scalability hard. Costly raw materials, lengthy processes, and difficulties in reproducibility hinder commercial production.
Additionally, limited understanding of the underlying mechanisms complicates designing better materials, while theoretical models struggle to predict stable, high-performance superconductors at ambient conditions.
How Does Pressure Affect the Superconducting Properties of These Materials?
You see, applying pressure can dramatically boost a superconductor’s shift temperature by changing its crystal structure and electronic states. Pressure reduces atomic spacing and alters bonding angles, which enhances electron pairing.
It can also suppress competing magnetic orders and improve charge mobility. By stabilizing these high-Tc phases through techniques like pressure quenching, you might keep their improved properties at ambient conditions, making practical applications more achievable.
Can High-Temperature Superconductors Operate Effectively in Ambient Magnetic Fields?
Think of high-temperature superconductors as resilient athletes, capable of performing in challenging environments. Yes, they operate effectively in ambient magnetic fields, thanks to their higher critical magnetic fields compared to traditional superconductors.
You can rely on them for applications like MRI machines and high-field magnets, where maintaining zero resistance under magnetic influence is essential. Their ability to withstand these fields makes them a versatile and promising technology for real-world use.
What Role Do Defects and Impurities Play in Superconducting Performance?
You should know that defects and impurities critically influence superconducting performance. They can lower the critical temperature by scattering electrons but also create pinning sites that trap vortices, improving current capacity.
However, too many defects, especially from radiation, cause degradation over time. Managing their type and concentration is essential, as they can either enhance or hinder superconductivity, depending on how you control their introduction and distribution.
Are There Any Naturally Occurring High-Temperature Superconducting Minerals?
You’re curious if any naturally occurring minerals act as high-temperature superconductors. Currently, only a few minerals, like covellite, are known to naturally exhibit superconductivity, but they usually do so at low temperatures.
Miassite is unique because it shows unconventional superconductivity with higher critical temperatures, hinting at natural high-temperature superconducting potential. These discoveries challenge previous beliefs and could pave the way for more sustainable, energy-efficient materials in the future.
Conclusion
Imagine holding a wire that’s perfectly frictionless—no resistance, just pure flow. That’s what high-temperature superconductors promise. They’ve revolutionized how we think about electricity, with some materials reaching transition temperatures above -135°C. Like a skilled chef blending ingredients seamlessly, scientists continue to refine their chemistry, pushing boundaries. As we gain access to more of this zero-resistance magic, the future of energy and technology becomes brighter—just like discovering a hidden pathway through a mountain.